Research on the applications of acoustic cavitation is frequently documented in relation to the characteristics within the spectrum of the emissions collected throughout the occurrence of cavitation.
Periodic shockwaves in acoustic cavitation measurements - CAVILUX laser illumination - Cavitar Ltd.
There is not a lot of knowledge available on the effects of particular bubble activity to spectral characteristics, further than a binary interpretation of stable compared to inertial cavitation.
In this investigation, laser-nucleation is utilized to commence cavitation within a few millimeters of a needle hydrophone’s tip, calibrated for magnitude and phase from 125 kHz to 20 MHz.
Acoustically driven at f0¼ 692 kHz, the bubble activity is presented with high-speed shadowgraphic imaging at 5 million frames per second. A synthetic spectrum is created from component signals founded on the hydrophone data, deconvolved within the calibration bandwidth, and in the time domain.
Cross correlation coefficients between the experimental and synthetic spectra of 0.97 for the f0/2 and f0/3 regimes suggest that scattered driving field and periodic shock waves are mainly responsible for all spectral features, such as the harmonics of f0, sub-harmonics and their over-harmonics.
Experimental Configuration
The experimental set-up displayed in Figure 1(a) is utilized to investigate cavitation in unrivaled detail, both acoustically and optically.
High-intensity focused ultrasound (HIFU) is emitted through a single element piezoceramic transducer (H-149, Sonic Concepts, Bothell, WA), joined to a power amplifier (2100L, Electronic and Innovation, Rochester, NY), and a waveform generator (DG4102, Rigol Technologies, Beijing, China).
The HIFU transducer holds a natural fundamental frequency of 200 kHz. For the investigation at hand, it is driven at the third harmonic through an impedance matching network, so that f0 = 692 kHz for all the results shown.
This driving frequency is selected so that acoustic cavitation emissions lie within the needle hydrophone’s calibration bandwidth (NH, 1.0 mm diameter, PVdF, Precision Acoustics, Dorchester, UK).
A central hole through the body of the transducer measuring 20 mm, functions to mount the NH, vertically aligned across the HIFU axis, Figure 1(a), with the tip positioned near the pre-focus -6 dB contour, ~3 mm from the focal point.
This orientation is referred to as the ‘emission collection’ position, as seen in Figure 1(b). The NH is fixed to an oscilloscope (MS07104A, Agilent Technologies, Lexington, MA).
.jpg)
Figure 1. Illustration of experimental setup: (a) cross-sectional side view, and (b) an axial scan of the HIFU focal region, with representations of the NH outlined for “emission collection”
To accurately begin cavitation activity in relation to the NH tip and in the HIFU focus, the laser enucleation method is employed.
A single 1.2 ± 0.1 mJ (instrument error regarding the manufacturer), 6 to 8 ns laser pulse (Nano S 130-10 frequency doubled Q-switched Nd:YAG, Litron Lasers, Rugby, UK), is moved through a long working distance microscope objective lens (50 0.42 NA Mitutoyo, Kawasaki, Japan), immersed in a sealed unit, fixed on an xyz manipulator (Velmex Motor, Bloomfield, NY), and is pre-aligned to the HIFU focus, ~3 mm above the tip of the NH in situ.
The laser pulse which was triggered to be incident ~5 cycles into a 65-cycle burst of HIFU, emitted the cavitation activity documented below. The transducer-NH configuration is kept within a tailor-made chamber, measuring 420 x 438 x 220 mm3 and filled with deionized, degassed water.
To enable the positioning of the imaging optics in proximity to the desired location of the cavitation, two of the walls of the chamber are recessed which allows sufficiently high spatial resolution imaging.
High-speed shadowgraphic imaging of the resulting cavitation activity is performed orthogonally to the nucleating laser axis, using a Monozoom 7 lens system (Bausch & Lomb, Rochester, NY), at 5 million frames per second (HPVX2, Shimadzu, Kyoto, Japan), with simultaneous 10 ns laser pulses (CAVILUX Smart, Cavitar, Tampere, Finland) offering effective illumination and temporal resolution in each frame.
A delay generator (DG535, Stanford Research Systems, Sunnyvale, CA) offers electronic triggering to coordinate all the instruments. The Q-switch of the laser, which is identified by the NH, signals cavitation nucleation and laser pulse emission, and is taken as t = 0 µs.
Results of High-Speed Imaging and Needle Hydrophone Data
Figures 2(a)–2(c) display the high-speed imaging data recorded for cavitation controlled by a PPPAHIFU = 1.63 ± 0.12 MPa.
The activity seems to comprise of a single bubble experiencing pseudo-spherical oscillation, with alternating powerful collapses concurrent to the shock wave generation at f0=2, captured Figure 2(b) at 8.25, 14.05, 17.05 µs and arrowed, Figure 2(c), and intervening partial deflations.
It is accepted that for the shadowgraphic imaging of acoustic transients, the focal plane for optimum resolution of the pressure variations is slightly removed from the plane where the generating bubble is positioned.
The bubble of Figures 2(a) and 2(b) is somewhat out of focus for this reason, and the bubble oscillation is not optimally resolved.
.jpg)
Figure 2. Images extracted from a high-speed sequence recorded at 5 mio fps, of cavitation activity in the f0/2 regime. (a) The whole field of view, depicting the NH tip position relative to the activity, with a shock wave (arrowed white) incident to it. (b) Selected images representing the cavitation oscillation dynamics, including three strong collapses, and coincident shock wave emission. The entire image sequence is available, in movie format, as supplementary material. (c) Radius-time curve based on a dark pixel counting algorithm, for the time interval under investigation. Diamond and squares indicate the specific images represented in (a) and (b), respectively. Scale bar represents 250 µm.
The results verify the cavitation behavior documented previously for a HIFU-cloud system in the f0=2 regime. Figure 2(c) graphically displays how the radius of the bubble varies over time, calculated from the whole image sequence recorded for this investigation.
Figure 3(a) is the raw voltage signal acquired in the emission collection position by the NH. A control experiment is also displayed where the HIFU burst was caused, but not the laser pulse incident to nucleate cavitation.
NH deconvolution within the calibration bandwidth and subtraction of the control makes up Figure 3. Figures 3(b) and 3(c) demonstrate the cavitation emission signal and the cavitation emission spectrum respectively.
There is a propagation time from every shock wave which is produced by the cloud and imaged using high-speed data acquisition, shown in Figure 2. Figure 3 shows the detection at the needle hydrophone of ~1.8 to 1.9 µs, according to the specific timing of the cloud collapse and the emission of the shock wave.
The propagation distance can be calculated from Figure 2(a) as ~2.7 mm but neither the location of the sensing element within the NH’s shaft, nor the features of the intervening material are known.
An average propagation speed of ~1520 ms–1 can be estimated from the high-speed imaging of periodic shockwave propagation, and a brief period of supersonic propagation may also be presumed.
.jpg)
Figure 3. (a) Raw NH data recorded during the cavitation activity of Figure 2 (blue solid) and control exposure for an equivalent HIFU burst (red dot), without cavitation nucleation. The inset zoom around 19.0 µs, reveals a detected shock wave in the raw data, also arrowed for the rest of the trace. (b) Control subtracted and NH-deconvolved data, revealing the signal emitted by the cavitation captured in the high-speed imaging of Figure 2. (c) The cavitation spectrum obtained via Fourier transformation of (b).
References and Further Reading
Deconvolution of acoustically detected bubble-collapse shock waves, Kristoffer Johansen, Jae Hee Song, Keith Johnston, Paul Prentice; Ultrasonics, Volume 73, January 2017, Pages 144–153.
Characterizing focused ultrasound via high speed shadowgraphic imaging at 10 million frames per second, Kristoffer Johansen, Jae Hee Song, Paul Prentice (CavLab, Cluster of Ultrasound Science, Technology and Engineering Research, University of Glasgow, UK); Ultrasonics Symposium (IUS), 2016 IEEE International, Date of Conference: 18-21 Sept. 2016.
Acknowledgments
Produced from materials originally authored by Jae Hee Song, Kristoffer Johansen, and Paul Prentice from the University of Glasgow.
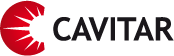
This information has been sourced, reviewed and adapted from materials provided by Cavitar.
For more information on this source, please visit Cavitar.