Severe acute respiratory coronavirus 2 (SARS-CoV-2) can be transmitted from one person to one or more people through viral transmission in airborne particles.
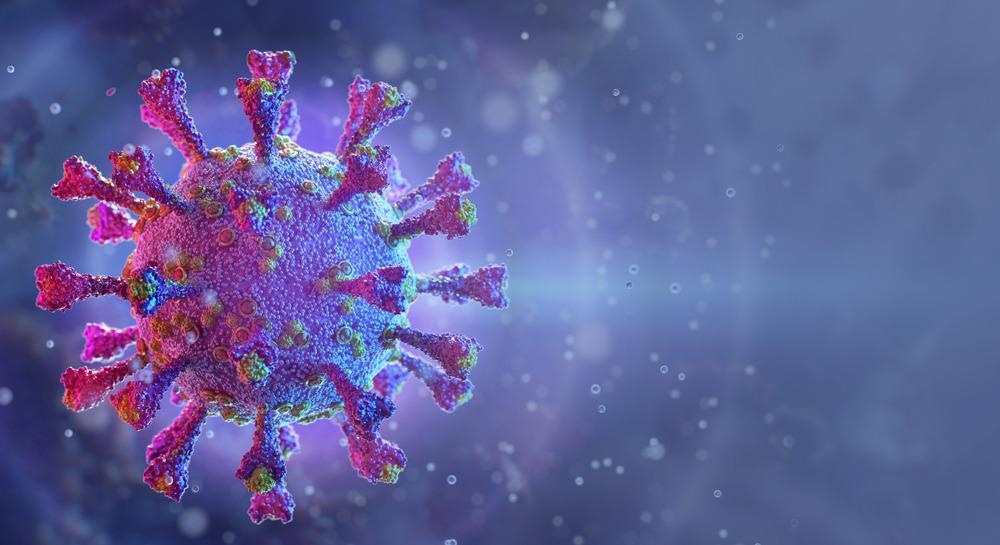
Image Credit: Corona Borealis Studio/Shutterstock.com
The high levels of SARS-CoV-2 transmission, as has been well documented, have overwhelmed national healthcare systems, resulting in millions of deaths and long-term health problems.
As a result, it is clear that reducing or eliminating SARS-CoV-2 transmission is a major and unprecedented global challenge. Transmission control measures have varying degrees of success and each has its own set of challenges. Although the evidence of vaccination’s efficacy in reducing disease transmission is unclear, it has been one of the most effective methods in minimizing death and serious illness.
Other than COVID-19, airborne transmission has been identified as a key mechanism for a variety of other viral infections, including measles, influenza, other human coronaviruses (Middle East Respiratory Syndrome MERS-CoV, SARS-CoV), and Respiratory Syncytial Virus (RSV), as well as bacterial infections such as tuberculosis and some pathogens responsible for hospital-acquired infections.
Germicidal ultraviolet (GUV) is a control measure that meets above the criteria and has a proven track record in the scientific community. Accidental human exposure to conventional 254 nm GUV, on the other hand, poses a significant challenge, as it can cause painful sunburn-like reactions in the skin and cornea.
“Far-UVC,” germicidal ultraviolet-C radiation with wavelengths ranging from 200 to 230 nm, is one possible solution, which are Krypton chloride (KrCl) excimer lamps. The primary emission wavelength is 222 nm. The low residual emission all through the ultraviolet region of the electromagnetic spectrum is a common source of Far-UVC.
KrCl excimer lamps have been shown to inactivate gram-negative and gram-positive bacteria, drug-resistant bacteria, human coronaviruses, including the SARS-CoV-2 virus, and influenza viruses in laboratory experiments.
While the laboratory results are promising, the inactivation of a pathogen in a restricted bench-scale laboratory environment does not always imply lowered disease transmission when the technology is used in the real world.
Experiments in huge, room-sized aerosol chambers are a translational step forward into real-world studies. This can also give insight into how to use technology in rooms where an infectious person is likely to be present for a long time.
The current research, for the first time, investigates the efficiency of Far-UVC for inactivating an airborne pathogen in a full-scale room-sized bioaerosol chamber under steady-state conditions.
Results
The ceiling of a room-sized bioaerosol chamber at the University of Leeds was secured with five Krypton Chloride excimer lamps, filtered to decrease ultraviolet emissions at wavelengths longer than 230 nm. The lamps were positioned in a quincunx pattern (Figure 1), with the emission aimed at the floor.
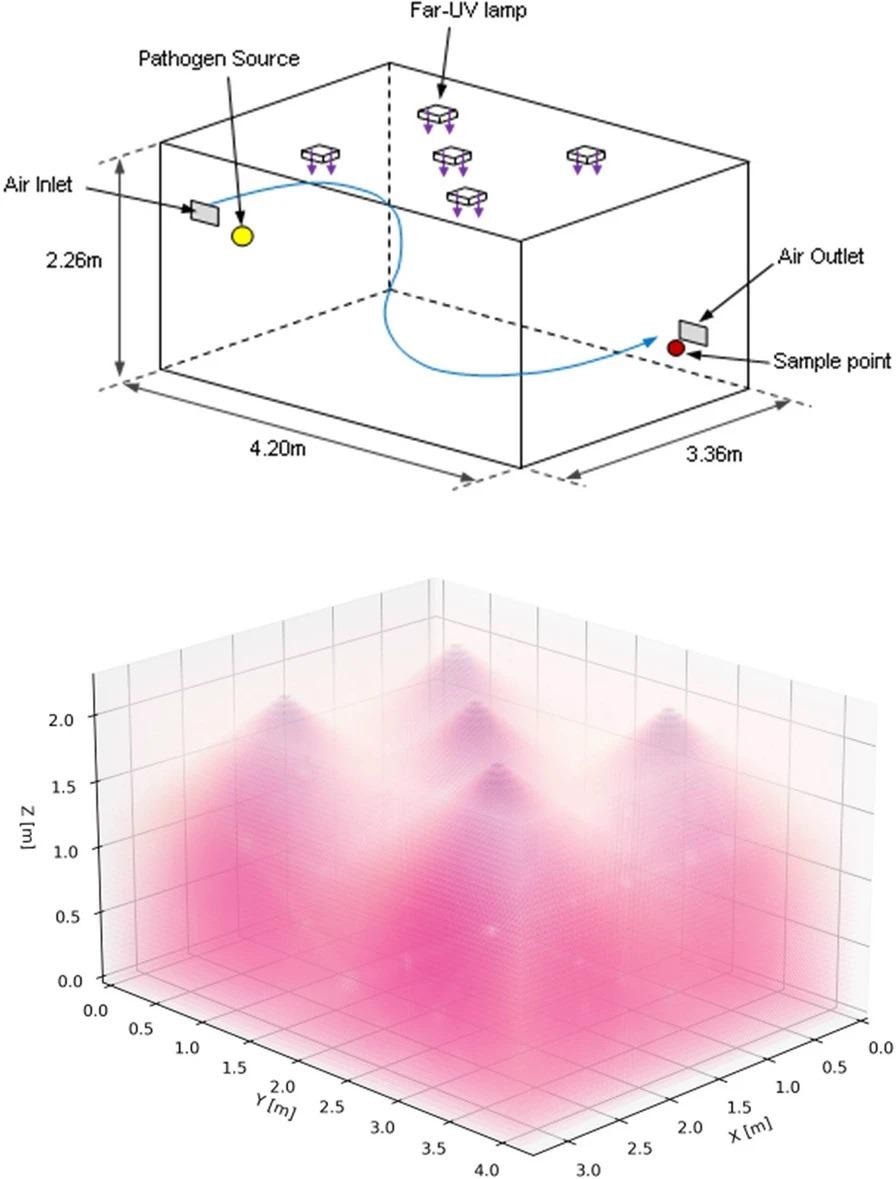
Figure 1. 3D schematics of the bioaerosol chamber configuration showing room dimensions, the position of the lamps, pathogen source, and collection point (top) with an illustrative example of the Far-UVC lamp emissions (bottom). Image Credit: Eadie, et al., 2022
A constant release of aerosolized Staphylococcus aureus has been introduced to the room at a height of 168 cm in a mechanically ventilated 32 m3 chamber. The rate of ventilation is three air changes per hour (ACH). Following a 60-minute stabilization period, 10 air samples were collected over 50 minutes. The sampling was then continued for another 50 minutes with either one or five Far-UVC sources turned on.
The evaluation was repeated three times with three distinct lamp exposure rates (Table 1). The International Commission on Non-Ionizing Radiation Protection (ICNIRP) guidelines for optical radiation exposure were used to determine the exposure rates.
Table 1. Average percentage pathogen reduction, irradiance, and calculated 8-hour exposure dose for three different exposure conditions at two heights from the ground. Source: Eadie, et al., 2022
|
Peak values |
Average values |
Average % pathogen reduction (SD) |
Height = 1.7 m |
Height = 1 m |
Height = 1.7 m |
Height = 1 m |
Irradiance
(µWcm-2) |
8-h dose
(mJcm-2) |
Irradiance
(µWcm-2) |
8-h dose
(mJcm-2) |
Irradiance
(µWcm-2) |
8-h dose
(mJcm-2) |
Irradiance
(µWcm-2) |
8-h dose
(mJcm-2) |
High |
1 lamp |
14.4 |
415 |
1.93 |
56 |
0.57 |
16.5 |
0.45 |
12.9 |
93.7**** (1.0) |
5 lamps |
14.4 |
415 |
3.42 |
98 |
2.73 |
78 |
2.01 |
58 |
98.4**** (0.7) |
Medium |
1 lamp |
0.92 |
26.5 |
0.13 |
3.7 |
0.03 |
0.87 |
0.03 |
0.82 |
65.9**** (4.0) |
5 lamps |
0.92 |
26.5 |
0.22 |
6.3 |
0.14 |
4.1 |
0.13 |
3.67 |
92.0**** (0.9) |
Low |
1 lamp |
0.09 |
2.65 |
0.01 |
0.37 |
0.003 |
0.09 |
0.003 |
0.08 |
12.8 ns (3.8) |
5 lamps |
0.09 |
2.65 |
0.02 |
0.63 |
0.01 |
0.41 |
0.01 |
0.37 |
28.7** (3.4) |
The bold, italicised 8-h exposure values are above the ICNIRP 222-nm exposure limit of 23 mJcm-2. No exposures exceeded the 2022 ACGIH threshold limit value for skin of 478 mJcm-2 at 222 nm. Statistical significance is represented by: ns = p > 0.05, * = p ≤ 0.05, ** = p ≤ 0.01, *** = p ≤ 0.001, and **** = p ≤ 0.0001.
Before and after the lamps were turned on, the concentration of viable S. aureus pathogens in the air at the collection location was serially sampled. Figure 2 and Table 1 show the results in colony-forming units per cubic meter (cfu m-3) for the 45 minutes prior to “lamp on,” as well as serially for the 50 minutes after “lamp on.”
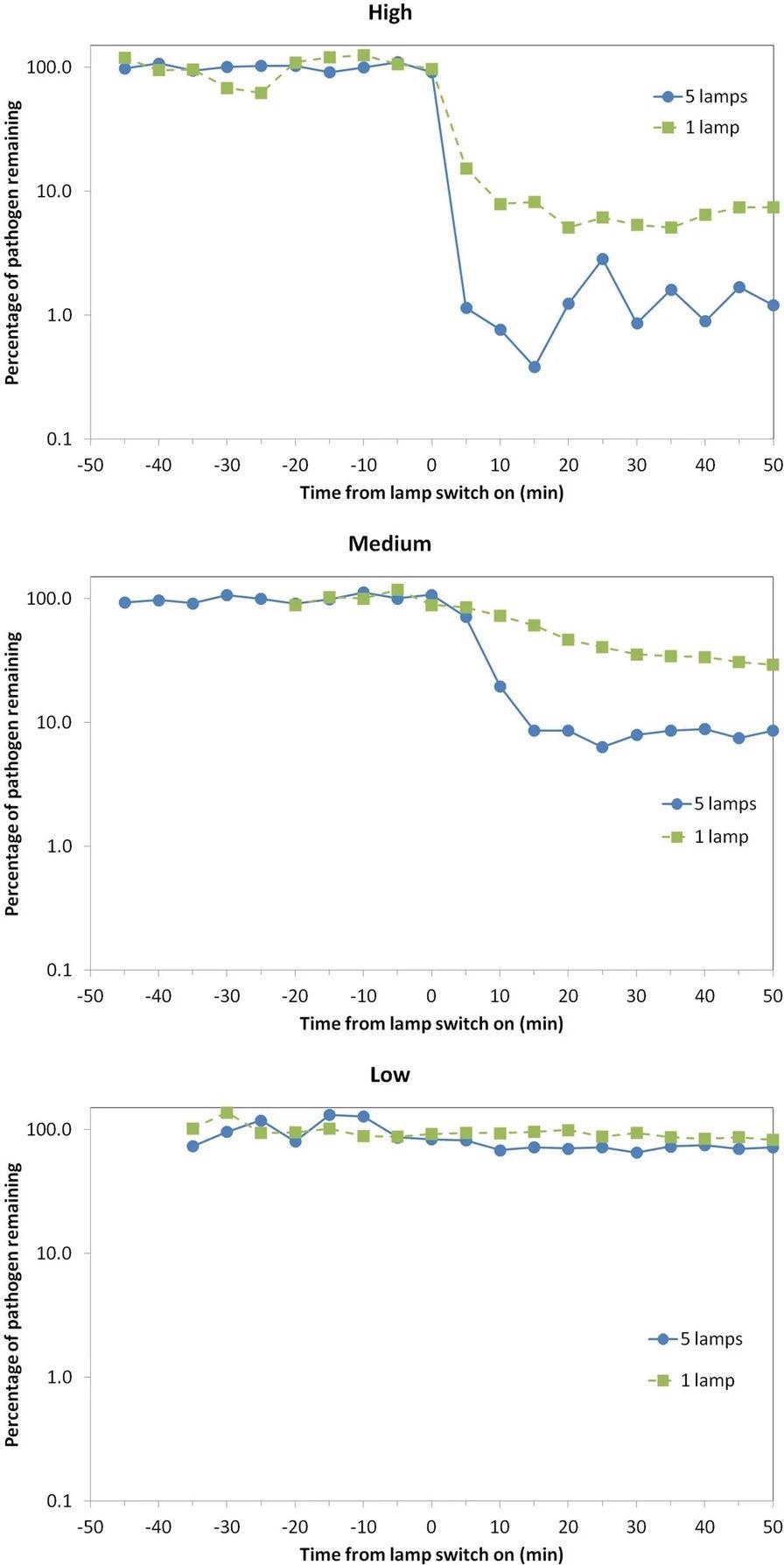
Figure 2. Percentage of viable airborne S. aureus remaining plotted on a logarithmic y-axis against time after switch-on of the Far-UVC sources for three different exposure scenarios—high (top), medium (middle), and low (bottom). Note that the pathogen was continuously released into the room throughout the experiment: The studies were undertaken using either a single central lamp (green, square data points, dashed lines) or all five Far-UVC lamps (blue, circular data points, solid lines). Image Credit: Eadie, et al., 2022
The “High” exposure scenario, as expected, resulted in the greatest reduction in the steady-state airborne viable S. aureus load. Despite the fact that the single lamp did not irradiate the entire room, the chamber’s good air mixing is likely to have resulted in this significant effect.
Using all five lamps, the “Medium” exposure scenario showed a 92% decrease in the steady-state viable pathogen load, with a maximum 8-hour exposure dose driven by the present ICNIRP guideline exposure limit at 222 nm of 23 mJcm-2.
The “Low” exposure scenario, which used very low intensity Far-UVC exposure rates, reduced viable pathogen load by 13% (one lamp) and 29% (five lamps).
Discussion
The study, published in Scientific Reports, displayed the capability of Far-UVC to rapidly reduce airborne pathogens in a realistically sized room with normal ventilation and a constant source of airborne pathogens for the first time. A 98% reduction was demonstrated at the ACGIH threshold limit values in less than 5 minutes. Figure 3 depicts a comparison of the two scenarios described.
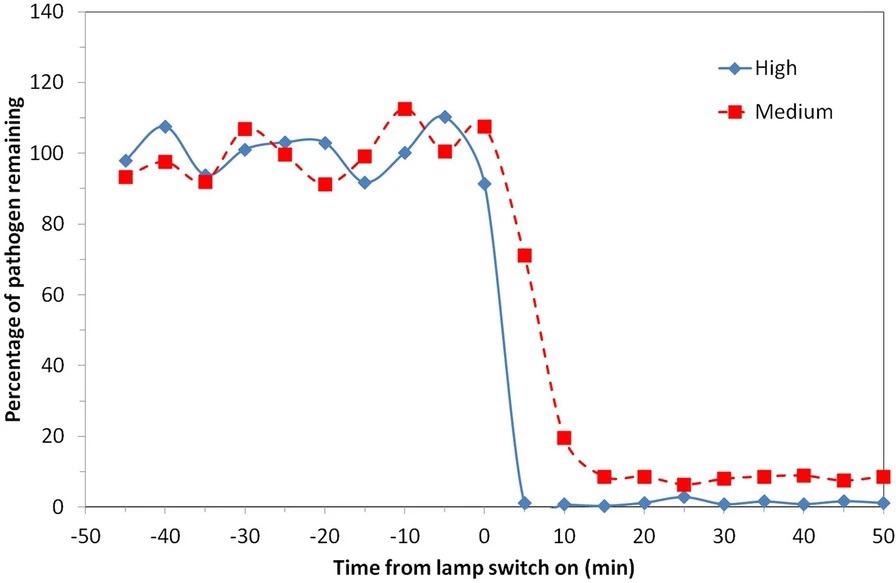
Figure 3. Percentage of viable airborne S. aureus remaining plotted on a linear y-axis for two of the exposure scenarios motivated by ICNIRP guideline exposure limits (5 lamps “Medium”) and ACGIH Threshold Limit Values (5 lamps “High”). Note that the pathogen was continuously released into the room throughout the experiment with a mechanical ventilation rate of 3 air changes per hour. Image Credit: Eadie, et al., 2022
Aerosolized S. aureus pathogen has been used as a surrogate for more relevant airborne viruses such as human coronaviruses and influenza viruses, despite the fact that SARS-CoV-2 was not used for safety reasons. The rationale for this can be seen in Figure 4, which compares Far-UVC inactivation rates of airborne influenza virus (H1N1), human coronaviruses (OC43 and 229E), and airborne S. aureus.
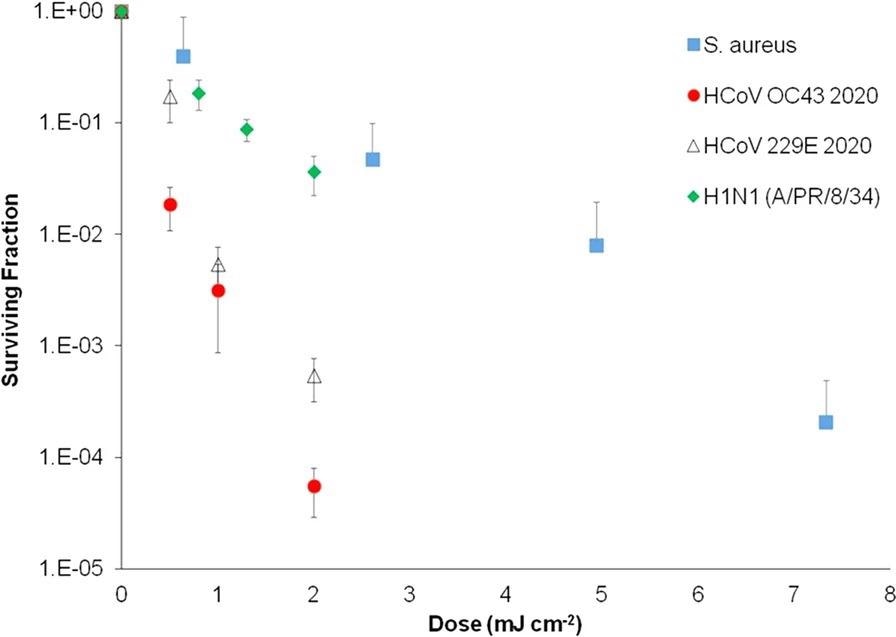
Figure 4. Inactivation of aerosolized human coronaviruses HCoV OC43 and HCoV 229E and H1N1 influenza virus at relevant low far-UVC doses, compared with aerosolized S. aureus with a Far-UVC lamp. Measurement taken at the Columbia University laboratory-based aerosolized pathogen irradiation system. HCoV OC43, HCoV 229E, and H1N1 influenza data were published previously and included for comparison. Image Credit: Eadie, et al., 2022
The findings show that airborne S. aureus is more resistant to Far-UVC inactivation than airborne influenza and human coronaviruses, implying that S. aureus is a conservative surrogate.
The peak lamp intensities in this study could have been five times higher than in the “Medium” scenario, improving inactivation while keeping the average 8-hour dose within ICNIRP guideline exposure limits.
This emphasizes the importance of proper Far-UVC installation to ensure that the designated space is properly and safely irradiated.
Methodology
Experiments were carried out in a bioaerosol chamber that was kept at a constant temperature. The chamber is mechanically ventilated and runs on negative pressure, with a HEPA-filtered fresh air system. It was important to avoid placing the bacteria release point and sample point directly under a Far-UVC source.
In practice, aerosolized level-3 pathogens such as SARS-CoV-2 could not be used in the bioaerosol chamber. S. aureus is also a pathogen of interest in and of itself because it is representative of Methicillin-resistant Staphylococcus aureus (MRSA), a pathogen that is common in hospital infections and is used as a standard for cleanliness.
It was also thought that assessing technology for pathogen inactivation well beyond the prevailing pandemic was crucial.
After irradiation on gelatin filters dissolved in 5 ml PBS, the airborne S. aureus was collected and inactivated using the colony-forming unit (CFU) assay on tryptone soy agar (TSA) plates.
Five commercially available Krypton Chloride excimer Far-UVC lamps were modified to include a diffusing material that broadened the Far-UVC distribution and increased the amount of irradiated volume.
A calibrated UVC radiometer was used to measure the irradiance (E) field in the chamber in the horizontal plane. Measurements were taken in 0.5 m intervals throughout the chamber at two heights (z) from the ground, 1.7 m and 1 m.
The production of aerosols was carried out in a controlled environment. Pathogen suspension in other materials (1% Foetal Bovine Serum) had no significant effect on the results, according to preliminary research.
An Anderson 6-stage impactor was used to collect the samples. After sampling, the agar plates were incubated for 24 hours at 37 °C. The number of colonies on each plate was then counted using the Gallenkamp colony counter.
Over the course of 60 minutes, the airborne Staphylococcus aureus was allowed to set up a steady-state within the chamber. The Far-UVC lamps were then turned on, and the sampling procedure was repeated.
Prior to turning on the Far-UVC devices, the concentrations of Staphylococcus aureus were normalized by equating them to the mean concentration of all samples to allow comparisons within and between experiments.
To compare viable pathogens before and 20 minutes after Far-UVC lamps were turned on, unpaired t-tests were used.
Conclusion
The findings provide some preliminary data for comparison with other technologies, such as portable air cleaners. Far-UVC may have an advantage over air cleaners and upper-room GUV in that it does not necessarily require “good” air mixing within the room. The researchers intend to work on this in the future.
More studies are needed to investigate the effects of variables such as humidity, temperature, proximity to the infectious source, and ventilation rates. However, the findings provide confidence that Far-UVC, when used appropriately and in accordance with the present (or future) safety regulatory limits, is likely to be an efficient, human behavior-independent control measure for inactivating key airborne pathogens such as human coronavirus and influenza.
Journal Reference
Eadie, E., Hiwar, W., Fletcher, L., Tidswell, E., O’Mahoney, P., Buonanno, M., Welch, D., Adamson, C. S., Brenner, D. J., Noakes, C., Wood, K. (2022) Far-UVC (222 nm) efficiently inactivates an airborne pathogen in a room-sized chamber. Scientific Reports, 12, p. 4373. Available Online: https://www.nature.com/articles/s41598-022-08462-z#citeas.
References and Further Reading
- Fennelly, K P., et al. (2020) Particle sizes of infectious aerosols: implications for infection control. The Lancet Respiratory Medicine, 8, 914–924. doi.org/10.1016/S2213-2600(20)30323-4.
- Santarpia, J. L. et al. (2020) Aerosol and surface contamination of SARS-CoV-2 observed in quarantine and isolation care. Scientific Reports, 10, pp. 1–8. doi.org/10.1038/s41598-020-69286-3.
- Greenhalgh, T. et al. Ten scientific reasons in support of airborne transmission of SARS-CoV-2. The Lancet (Lond. Engl.) 397, pp. 1603–1605. doi.org/10.1016/S0140-6736(21)00869-2.
- Morawska, L. & Milton, D. K. It is time to address airborne transmission of coronavirus disease 2019 (COVID-19). Clinical Infectious Diseases, 71, pp. 2311–2313. doi.org/10.1093/cid/ciaa939.
- Miller, S L. et al. (2021) Transmission of SARS-CoV-2 by inhalation of respiratory aerosol in the Skagit Valley Chorale superspreading event. Indoor Air, 31, pp. 314–323. doi.org/10.1111/ina.12751.
- World Health Organisation (2021) Roadmap to improve and ensure good indoor ventilation in the context of COVID-19. https://www.who.int/publications/i/item/9789240021280.
- Harder, T. et al. (2021) Efficacy and effectiveness of COVID-19 vaccines against SARS-CoV-2 infection: interim results of a living systematic review, 1 January to 14 May 2021. Eurosurveillance, 26, p. 2100563.
- Hetemäki, I. et al. (2021) An outbreak caused by the SARS-CoV-2 Delta variant (B.1.617.2) in a secondary care hospital in Finland, May 2021. Eurosurveillance, 26, p. 2100636.
- Rader, B. et al. (2021) Mask-wearing and control of SARS-CoV-2 transmission in the USA: a cross-sectional study. The Lancet Digital Health, 3, pp. e148–e157. https://doi.org/10.1016/S2589-7500(20)30293-4.
- Howard, J. et al. (2021) An evidence review of face masks against COVID-19. Proceedings of the National Academy of Sciences of the United States of America, p. 118. doi.org/10.1073/pnas.2014564118.
- Personal measures taken to avoid COVID-19 |YouGov. https://yougov.co.uk/topics/international/articles-reports/2020/03/17/personal-measures-taken-avoid-covid-19.
- World Health Organisation Regional Office for Europe. (2020) Pandemic fatigue Reinvigorating the public to prevent COVID-19. Policy framework for supporting pandemic prevention and management. http://apps.who.int/bookorders.
- Wang, C. C. et al. (2021) Airborne transmission of respiratory viruses. Science, 373, p. 80. doi.org/10.1126/science.abd9149.
- Tang, J. W., et al. (2015) Aerosol-transmitted infections—a new consideration for public health and infection control teams. Current Treatment Options in Infectious Diseases. 7, 176–201. doi.org/10.1007/s40506-015-0057-1.
- Escombe, A. R. et al. (2009) Upper-room ultraviolet light and negative air ionization to prevent tuberculosis transmission. PLoS Med. 6, p. e1000043. doi.org/10.1371/journal.pmed.1000043.
- Wells, W. F., et al. (1942) The environmental control of epidemic contagion: I. An epidemiologic study of radiant disinfection of air in day schools. American Journal of Epidemiology, 35, pp. 97–121. doi.org/10.1093/oxfordjournals.aje.a118789.
- International Commission on Non-Ionizing Radiation Protection. ICNIRP Guidelines on limits of exposure to ultraviolet radiation of wavelengths between 180 nm and 400 nm (Incoherent Optical Radiation). Health Physics, 87, pp. 171–186.
- Nardell, E. A. et al. Safety of upper-room ultraviolet germicidal air disinfection for room occupants: results from the Tuberculosis Ultraviolet Shelter Study. Public Health Reports. 123, pp. 52–60. doi.org/10.1177/003335490812300108.
- Lyons, A B & Hamzavi, I H (2020) Ultraviolet C induced skin reaction from ultraviolet germicidal irradiation of N95 respirators during the COVID-19 pandemic. Photodermatology, Photoimmunology and Photomedicine, 37, pp. 159–160. doi.org/10.1111/phpp.12612.
- Trevisan, A. et al. (2006) Unusual high exposure to ultraviolet-C radiation. Photochemistry and Photobiology, 82, p. 1077. doi.org/10.1562/2005-10-27-ra-728.
- Eadie, E., et al. (2021) Extreme exposure to filtered far-UVC: a case study. Photochemistry and Photobiology, 97, pp. 527–531. doi.org/10.1111/php.13385.
- Kitagawa, H. et al. (2020) Effectiveness of 222-nm ultraviolet light on disinfecting SARS-CoV-2 surface contamination. American Journal of Infection Control, 49, pp. 299–301. doi.org/10.1016/j.ajic.2020.08.022.
- Narita, K. et al. (2020) Ultraviolet C light with wavelength of 222 nm inactivates a wide spectrum of microbial pathogens. Journal of Hospital Infection, 105, pp. 459–467. https://doi.org/10.1016/j.jhin.2020.03.030.
- Matafonova, G. G. G., et al. (2008) Efficiency of KrCl excilamp (222 nm) for inactivation of bacteria in suspension. Letters in Applied Microbiology, 47, pp. 508–513. doi.org/10.1111/j.1472-765x.2008.02461.x.
- Wang, D., et al. (2010) Comparison of the disinfection effects of vacuum-UV (VUV) and UV light on bacillus subtilis spores in aqueous suspensions at 172, 222 and 254 nm. Photochemistry and Photobiology, 86, pp. 176–181. doi.org/10.1111/j.1751-1097.2009.00640.x.
- Buonanno, M. et al. (2017) Germicidal efficacy and mammalian skin safety of 222-nm UV light. Radiation Research. 187, pp. 493–501. doi.org/10.1667/rr0010cc.1.
- Buonanno, M., et al. (2020) Far-UVC light (222 nm) efficiently and safely inactivates airborne human coronaviruses. Scientific Reports, 10, pp. 1–8. doi.org/10.1038/s41598-020-67211-2.
- Welch, D. et al. (2018) Far-UVC light: a new tool to control the spread of airborne-mediated microbial diseases. Scientific Reports, 8, pp. 1–7. doi.org/10.1038/s41598-018-21058-w.
- Hickerson, R. P., et al. (2021) Minimal, superficial DNA damage in human skin from filtered far-ultraviolet-C (UV-C). British Journal of Dermatology, 184, pp. 1197–1199. doi.org/10.1111/bjd.19816.
- Narita, K., et al. (2018) Chronic irradiation with 222-nm UVC light induces neither DNA damage nor epidermal lesions in mouse skin, even at high doses. PLoS ONE, 13, p. e0201259. doi.org/10.1371/journal.pone.0201259.
- Kaidzu, S. et al. (2019) Evaluation of acute corneal damage induced by 222-nm and 254-nm ultraviolet light in Sprague-Dawley rats. Free Radical Research, 53, pp. 611–617. doi.org/10.1080/10715762.2019.1603378.
- Kaidzu, S. et al. (2021) Re-evaluation of rat corneal damage by short wavelength UV revealed extremely less hazardous property of far-UV-C. Photochemistry and Photobiology, 97, pp. 505–516. doi.org/10.1111/php.13419.
- Yamano, N. et al. (2020) Long-term effects of 222 nm ultraviolet radiation C sterilizing lamps on mice susceptible to ultraviolet radiation. Photochemistry and Photobiology, 94, pp. 853–862. https://doi.org/10.1111/php.13269.
- Fukui, T. et al. (2020) Exploratory clinical trial on the safety and bactericidal effect of 222-nm ultraviolet C irradiation in healthy humans. PLoS ONE, 15, e0235948. doi.org/10.1371/journal.pone.0235948
- Nardell, E. & Nathavitharana, R (2019) Air disinfection in measles transmission hotspots. Lancet, 394, pp. 1009–1010. doi.org/10.1016/S0140-6736(19)31889-6.
- Memarzadeh, F., et al. (2010) Applications of ultraviolet germicidal irradiation disinfection in health care facilities: Effective adjunct, but not stand-alone technology. American Journal of Infection Control, 38, pp. S13–S24. doi.org/10.1016/j.ajic.2010.04.208.
- Wells, W. F (1943) Air disinfection in day schools. American Journal of Public Health and the Nation’s Health, 33, pp. 1436–1443. doi.org/10.2105/ajph.33.12.1436.
- Hathway, E. A., et al. (2011) CFD simulation of airborne pathogen transport due to human activities. Building and Environment, 46, pp. 2500–2511. https://doi.org/10.1016/j.buildenv.2011.06.001.
- King, M.-F., et al. (2017) An effective surrogate tracer technique for S. aureus bioaerosols in a mechanically ventilated hospital room replica using dilute aqueous lithium chloride. Atmosphere, 8, p. 238. doi.org/10.3390/atmos8120238.
- King, M. F., et al. (2013) Bioaerosol deposition in single and two-bed hospital rooms: a numerical and experimental study. Building and Environment, 59, pp. 436–447. doi.org/10.1016/j.buildenv.2012.09.011.
- Gilkeson, C. A. & Noakes, C (2013) Application of CFD Simulation To Predicting Upper-Room UVGI effectiveness. Photochemistry and Photobiology, 89, pp. 799–810. doi.org/10.1111/php.12013.
- Beggs, C. B., et al. (2006) Methodology for determining the susceptibility of airborne microorganisms to irradiation by an upper-room UVGI system. Journal of Aerosol Science, 37, pp. 885–902. doi.org/10.1016/j.jaerosci.2005.08.002.
- Xu, P. et al. (2003) Efficacy of ultraviolet germicidal irradiation of upper-room air in inactivating airborne bacterial spores and mycobacteria in full-scale studies. Atmospheric Environment, 37, pp. 405–419. doi.org/10.1016/S1352-2310(02)00825-7.
- ACGIH. 2022 TLVs and BEIs. (2022)
- Kitagawa, H. et al. (2021) Effect of intermittent irradiation and fluence-response of 222 nm ultraviolet light on SARS-CoV-2 contamination. Photodiagnosis and Photodynamic Therapy, 33, p. 102184. doi.org/10.1016/j.pdpdt.2021.102184.
- Ma, B., et al. (2021) UV Inactivation of SARS-CoV-2 across the UVC spectrum: KrCl excimer, mercury-vapor, and LED sources. Applied and Environmental Microbiology, doi.org/10.1128/AEM.01532-21.
- Ma, B. et al. (2021) Inactivation of Coronaviruses and Phage Phi6 from Irradiation across UVC Wavelengths. Environmental Science & Technology Letters, doi.org/10.1021/acs.estlett.1c00178.
- First, M. W., et al. (2005) Monitoring human exposures to upper-room germicidal ultraviolet irradiation. Journal of Occupational and Environmental Hygiene, 2, pp. 285–292. doi.org/10.1080/15459620590952224.
- Wood, K., et al. (2021) Turn up the lights, leave them on and shine them all around—numerical simulations point the way to more efficient use of far-UVC lights for the inactivation of airborne coronavirus. Photochemistry and Photobiology, doi.org/10.1111/php.13523.
- Macher, J M (1989) Positive-hole correction of multiple-jet impactors for collecting viable microorganisms. American Industrial Hygiene Association Journal, 50, pp. 561–568. doi.org/10.1080/15298668991375164