Due to its unusual structure and exceptional characteristics, graphene has sparked significant interest in academic and industry circles since its discovery in 2004. Notably, chemical vapor deposition (CVD) technology has increased the commercialization and industrialization of CVD graphene-based applications such as biosensing, ion sieving, and supporting membrane for transmission electron microscopy (TEM) imaging.
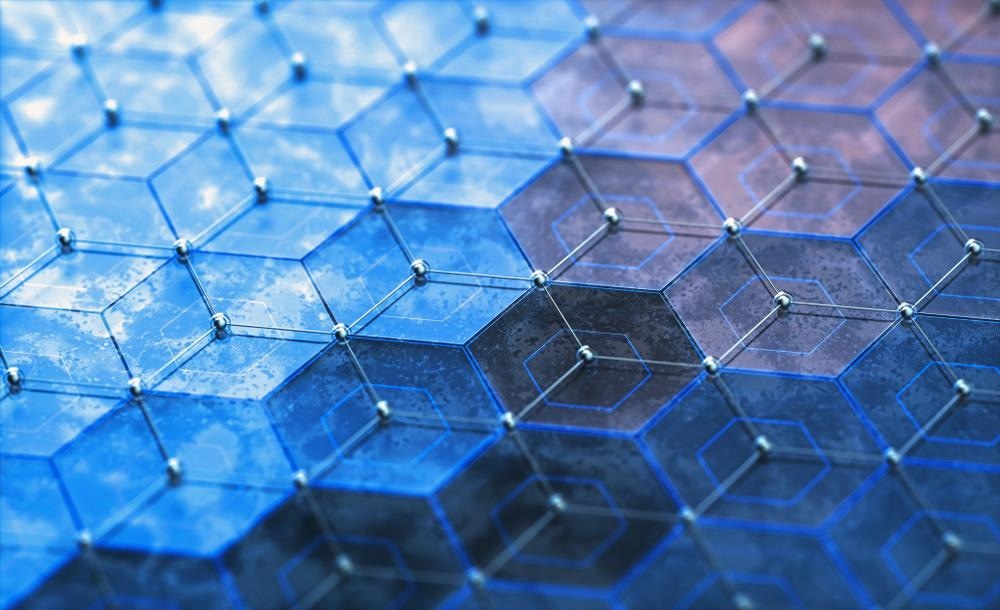
Image Credit: ktsdesign/Shutterstock.com
This article looks at research conducted and published in Nano Letters.
The interaction of graphene with water is critical in many applications, and graphene with high hydrophilicity would be ideal. As a result, controlled alteration of graphene’s water wettability on functional substrates, particularly CVD-produced graphene, is critical for application performance and reliability.
The static water contact angle (WCA) measurement methodology has been extensively used to assess the wettability of CVD graphene films and other two-dimensional (2D) materials, such as hBN, on growth substrates or functional substrates, such as SiO2/Si, quartz, or polymer following transfer.
In contrast, multiple recent investigations have indicated that new graphene surfaces are naturally hydrophilic and that the wettability of graphene is very reactive to its surroundings, particularly surface contamination, which would cause the graphene surface to become hydrophobic.
Meanwhile, even for the hydrophilic graphene surface, the published WCA values are still spread out, implying that the major mechanisms governing graphene wettability are unknown. Because graphene’s wettability is very susceptible to surface contamination, the newly discovered intrinsic contamination, amorphous carbon, which forms on the graphene surface during CVD development, would also affect graphene’s surface characteristics.
As a result, eliminating the inherent contamination would allow for the direct production of hydrophilic CVD graphene for practical applications such as TEM imaging and cell culture.
The hydrophilic nature of clean graphene films straight after CVD formation on single-crystal Cu (111) substrate was confirmed in this study, with an average WCA of ∼23°. Both experimental and theoretical research was used to evaluate the influence of amorphous carbon contamination on graphene wettability.
The wettability of as-transferred graphene over quartz substrate is significantly reliant on its surface cleanness; hence, clean graphene with a hydrophilic surface performed better as a cell culture plate support. Similarly, when used as a supportive membrane for cryoelectron microscopy (cryo-EM) imaging, the suspended pure graphene allowed for high-density biomolecule filling for three-dimensional (3D) reconstruction.
This research not only clarifies CVD graphene’s inherent hydrophilicity but also opens up new possibilities for CVD graphene-based bio applications.
Result
The impact of inherent surface contamination, i.e., amorphous carbon, just on water wettability of CVD-grown graphene on Cu was widely studied using static WCA measurements (Figure 1a). The amorphous carbon contamination (dark contrast) nearly occupied approximately 40% of the area in traditionally generated graphene, as shown in Figure 1(b), and continuous clean patches were no more than 100 nm in size.
After the CO2 treatment, graphene was visible in the TEM image with a uniform and stronger contrast (Figure 1c), suggesting that surface contamination on a graphene surface was already completely etched away by the CO2.
The clean graphene had a hydrophilic nature with an overall WCA of ∼35°, but the unclean graphene had an overall WCA of ∼64°, as illustrated in Figure 1(d). The WCAs of both the clean and unclean graphene grew substantially during the first 10 minutes after being removed from the growing chamber, as seen in Figure 1(e). Following that, after 20 minutes of exposure, WCA readings steadily grew until they reached their maximum value.
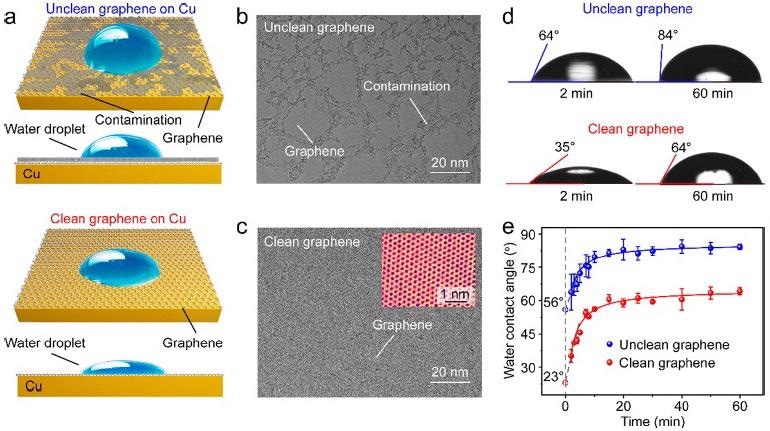
Figure 1. Wettability of CVD-derived unclean and clean graphene on Cu (111) substrates. (a) Schematic illustration of the impact of surface contamination on the wettability of graphene/Cu. (b, c) TEM images of the unclean (b) and clean (c) graphene. Inset in (c): High-resolution TEM image of the clean graphene with atomic resolution. (d) WCAs of the unclean (top) and clean (down) graphene/Cu after they were taken out of the CVD chamber for 2 minutes (left) and 60 minutes (right). (e) Time evolution of the WCAs of the Cu-supported unclean (blue) and clean (red) graphene after exposure in ambient air for 60 minutes. Image Credit: Zhang, et al., 2021
The WCA of clean graphene/Cu (111) was estimated to be ∼21° (Figure 2a), which matches the actual result. Surface contamination on the graphene/Cu surface would raise WCA to ∼58° (Figure 2b), making the unclean graphene lesser hydrophilic. Similarly, when the contaminated area ratio grew from 0–50%, the estimated WCA values increased from ∼21–∼75° (Figure 2c).
The most stable adsorption arrangement of water molecules can be confirmed by finding the minimum ground state energy of the molecule of water adsorbed on the clean graphene/Cu (111) surface (Figure 2d, e), where the spacing between both the oxygen atom of the water molecule and the graphene plane (such that the height of the water molecule) was 0.314 nm with an adsorption energy of -166.95 meV.
The adsorption energies of water molecules on four different locations above the contamination were then estimated by altering the horizontal distances between water molecules and the center of the amorphous carbon contamination, and the results ranged from -110 to -93 meV. (Figure 2f, g).
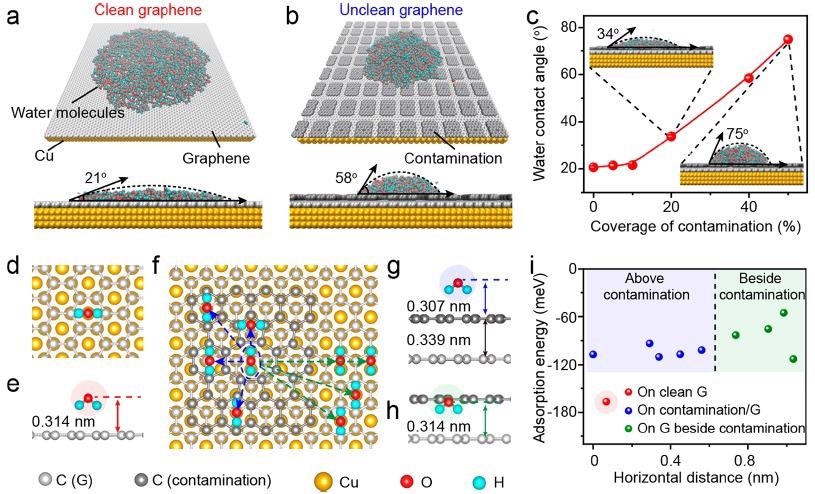
Figure 2. Theoretical analysis of the water wettability on graphene/Cu. (a, b) Representative MD simulation results of the water molecules distribution on the clean (a) and unclean (b) (with contamination coverage of 40%) graphene/Cu (111) surface. (c) Relationship between the WCA values of graphene and the coverage of surface contamination. Inset: MD simulation results of the unclean graphene with the contamination coverage of 20% (up left) and 50% (bottom right). (d, e) Top (d) and side (e) views of the water molecule adsorbed atop clean graphene surface. (f) Top view of the water molecules adsorbed on the surface of the contaminated graphene with varied horizontal distance. (g, h) Representative side views of the water molecule adsorbed above the contamination (g) and above graphene beside the contamination (h). (i) Relationship between the adsorption energy of the water molecules and the horizontal distance from O atom in the water molecule to the center of the amorphous carbon contamination. Image Credit: Zhang, et al., 2021
The wettability of the cm-sized as-transferred clean and unclean graphene samples was varied due to the varying level of surface contamination (Figure 3a). The average WCA for clean and unclean graphene is ∼40° and ∼70°, respectively (Figure 3b-d), indicating that the hydrophilic character of clean graphene is preserved after transfer.
The biocompatibility of the transferred graphene, as well as the influence of its surface cleanness and wettability on the cellular proliferation, were assessed using both clean graphene and unclean graphene over quartz substrates as plate supports for cell culture (Figure 3e).
The number of cells cultivated on the clean graphene surface was larger than with the unclean graphene surface (Figure 3f, g), which may be related to the enhanced wettability and increased surface flatness of the clean graphene, indicating superior biocompatibility.
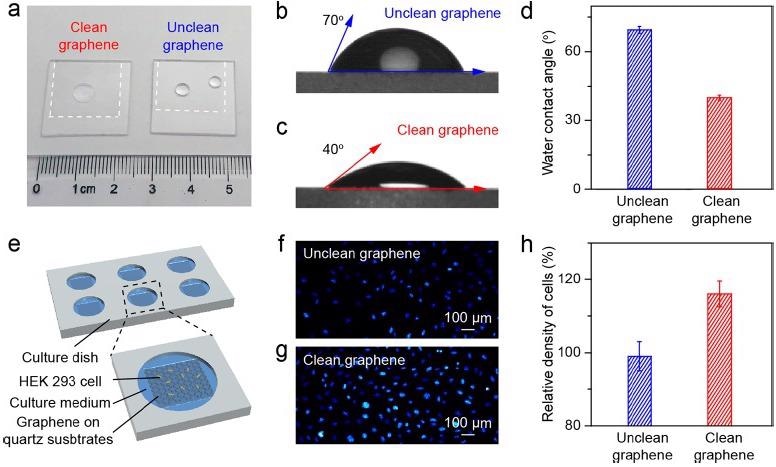
Figure 3. Application of the clean graphene in cell culture. (a) Photograph of water droplets on the clean (left) and unclean (right) graphene supported by the quartz substrates. (b, c) WCA measurement results of the as-transferred unclean (b) and clean (c) graphene on quartz substrates. (d) Statistical results of the WCA values of the unclean (blue) and clean (red) graphene on quartz substrates. (e) Schematic illustration of the cell culture using graphene as the culture plate support. (f, g) Fluorescence microscope images of cultured cells on the unclean (f) and clean (g) graphene surface after proliferation for 48 hours. (h) Comparison of the density of cells cultured on the unclean (blue) and clean (red) graphene on quartz substrates, using the cell density on the bare quartz substrate as a reference. Image Credit: Zhang, et al., 2021
On the suspending graphene membrane, cryoenvironmental scanning EM (ESEM) and cryo-TEM characterizations were performed to assess the influence of surface contamination on the regional distribution of water over graphene surfaces (Figure 4a).
Figure 4b shows a continuous water membrane with homogeneous contrast produced on the clean graphene surface, whereas Figure 4c shows high-density spherical-shaped droplets of water on the unclean graphene surface, illustrating clean graphene’s improved wettability.
On the clean graphene surface (Figure 4d), a high-density and homogeneous distribution of PNPase particles in the unified ice layer was visible, whereas few PNPase particles were visible on the unclean graphene surface, which was similar to previous results obtained using conventional graphene growth and transfer methods (Figure 4e).
PNPase particles took on a variety of configurations on the suspended clean graphene membrane (Figure 4f), allowing for a credible 3D cryo-EM reconstruction of PNPase with well-defined secondary structural information (Figure 4g, h).
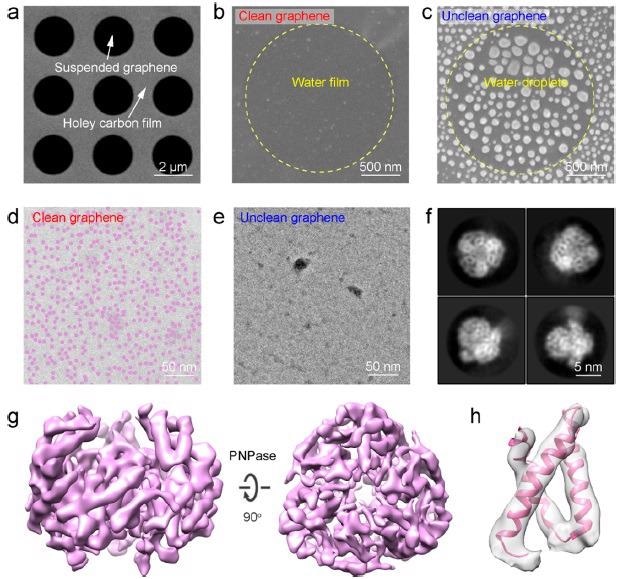
Figure 4. Application of the clean graphene on cryo-EM imaging. (a) Scanning EM (SEM) image of the large-area suspended graphene membrane. (b, c) SEM images showing the distribution of water on the surface of the suspended clean (b) and unclean (c) graphene. (d, e) TEM images showing the distribution of ice layer and PNPase particles on the clean (d) and unclean (e) graphene. Note that PNPase particles are dyed in semitransparent purple color in (d). (f) Representative 2D class averages of PNPase supported by the clean graphene. (g) 3D reconstruction map of the PNPase particle using the clean graphene as the supporting substrate. (h) Selected region of PNPase’s map with the corresponding model docked. Image Credit: Zhang, et al., 2021
Conclusion
By using both experimental and theoretical analyses, this paper establishes the hydrophilic nature of pure graphene and highlights the influence of intrinsic contamination on graphene wettability. Additionally, after being placed onto functional substrates, clean graphene has higher hydrophilic properties than unclean graphene, providing clear benefits for cell culture and cryo-EM imaging applications.
This research not only confirms graphene’s inherent hydrophilicity but also sheds light on how to build innovative applications based on CVD graphene with a clean surface.
Journal Reference
Zhang, J., Jia, K., Huang, Y., Wang, Y., Liu, N., Chen, Y., Liu, X., Liu, X., Zhu, Y., Zheng, L., and Chen, H. (2021). Hydrophilic, Clean Graphene for Cell Culture and Cryo-EM Imaging. Nano Letters, 21(22), pp.9587-9593. Available Online: https://pubs.acs.org/doi/10.1021/acs.nanolett.1c03344?ref=pdf.
References and Further Reading
- Ye, R & Tour, J M (2019) Graphene at fifteen. ACS Nano, 13(10), pp. 10872−10878.doi.org/10.1021/acsnano.9b06778.
- Kong, W., et al. (2019)Path towards graphene commercialization from lab to market. Nature Nanotechnology, 14, pp. 927−938.doi.org/10.1038/s41565-019-0555-2.
- Lin, L., et al. (2019) Synthesis challenges for graphene industry. Nature Materials, 18, pp. 520−524.doi.org/10.1038/s41563-019-0341-4.
- Zhou, K. G., et al. (2018) Electrically controlled water permeation through graphene oxide membranes. Nature, 559, pp. 236−240.doi.org/10.1038/s41586-018-0292-y.
- Keerthi, A., et al. (2018) Ballistic molecular transport through two-dimensional channels. Nature, 558, pp. 420−424.doi.org/10.1038/s41586-018-0203-2.
- Feng, J&Guo, Z (2019) Wettability of graphene: from influencing factors and reversible conversions to potential applications. Nanoscale Horizons, 4, pp. 339−364.doi.org/10.1039/C8NH00348C.
- Snapp, P., et al. (2020) Interaction of 2D materials with liquids: wettability, electrochemical properties, friction, and emerging directions. NPG Asia Materials, 12(1), p. 22.doi.org/10.1038/s41427-020-0203-1.
- Wang, J., et al. (2018) Programmable wettability on photocontrolled graphene film. Science Advances, 4(9), p. eaat7392.doi.org/10.1126/sciadv.aat7392.
- Melios, C., et al. (2018) Water on graphene: review of recent progress. 2D Materials, 5(2), p. 022001.doi.org/10.1088/2053-1583/aa9ea9.
- Belyaeva, L A & Schneider, G F (2020) Wettability of graphene. Surface Science Reports, 75(2), p. 100482.doi.org/10.1016/j.surfrep.2020.100482
- Li, X., et al. (2017) Wettability of supported monolayer hexagonal boron nitride in air. Advanced Function Materials, 27(19), p. 1603181.doi.org/10.1002/adfm.201603181.
- Raj, R., et al. (2013) Wettability of graphene. Nano Letters, 13(4), pp. 1509−1515.doi.org/10.1021/nl304647t.
- Hong, G., et al. (2016) On the Mechanism of hydrophilicity of graphene. Nano Letters, 16(7), pp. 4447−4453.doi.org/10.1021/acs.nanolett.6b01594.
- Ashraf, A., et al. (2016) Doping-Induced tunable wettability and adhesion of graphene. Nano Letters, 16(7), pp. 4708–4712.doi.org/10.1021/acs.nanolett.6b02228.
- Kozbial, A., et al. (2017) Characterization of the intrinsic water wettability of graphite using contact angle measurements: effect of defects on static and dynamic contact angles. Langmuir, 33(4), pp. 959−967.doi.org/10.1021/acs.langmuir.6b04193.
- Liu, N., et al. (2019) Bioactive functionalized monolayer graphene for high-resolution Cryo-Electron microscopy. Journal of the American Chemical Society, 141(9), pp. 4016−4025.doi.org/10.1021/jacs.8b13038.
- Son, J., et al. (2020) Tunable wettability of graphene through nondestructive hydrogenation and wettability-based patterning for bioapplications. Nano Letters, 20(8), pp. 5625−5631.doi.org/10.1021/acs.nanolett.9b04548.
- Li, Z., et al. (2013) Effect of airborne contaminants on the wettability of supported graphene and graphite. Nature Materials, 12, pp. 925−931.doi.org/10.1038/nmat3709.
- Prydatko, A. V., et al. (2018) Contact angle measurement of free-standing squaremillimeter single-layer graphene. Nature Communications, 9, p. 4185.doi.org/10.1038/s41467-018-06608-0.
- Belyaeva, L. A., et al. (2018) Hydrophilicity of graphene in water through transparency to polar and dispersive interactions. Advanced Materials, 30(6), p. 1703274.doi.org/10.1002/adma.201703274.
- Zheng, L. M., et al. (2020) Robust ultraclean atomically thin membranes for atomic-resolution electron microscopy. Nature Communications, 11, p. 541.doi.org/10.1038/s41467-020-14359-0.
- Russo, C J & Passmore, L A (2014) Controlling protein adsorption on graphene for cryo-EM using low-energy hydrogen plasmas. Nature Methods, 11, pp. 649–652.doi.org/10.1038/nmeth.2931.
- Xu, Z., et al. (2015) Reversible hydrophobic to hydrophilic transition in graphene via water splitting induced by UV irradiation. Science Report, 23(4), p. 6450.doi.org/10.1038/srep06450.
- Zheng, L., et al. (2020) Robust ultraclean atomically thin membranes for atomic-resolution electron microscopy. Nature Communications, 11, p. 541.doi.org/10.1038/s41467-020-14359-0.
- Zhang, J., et al. (2020) New growth frontier: superclean graphene. ACS Nano, 14(9), pp. 10796−10803.doi.org/10.1021/acsnano.0c06141.
- Lin, L., et al. (2019) Towards super-clean graphene. Nature Communications, 10, p. 1912.doi.org/10.1038/s41467-019-09565-4.
- Zhang, J., et al. (2019) Large-area synthesis of superclean graphene via selective etching of amorphous carbon with carbon dioxide. Angewandte Chemie International Edition, 58(41), pp. 14446−14451.doi.org/10.1002/anie.201905672.
- Zhang, J., et al. (2017) Clean transfer of large graphene single crystals for high-intactness suspended membranes and liquid cells. Advanced Materials, 29(26), p. 1700639.doi.org/10.1002/adma.201700639.
- Kozbial, A., et al. (2016) Are graphitic surfaces hydrophobic? Accounts of Chemical Research, 49(12), pp. 2765−2773.doi.org/10.1021/acs.accounts.6b00447.
- Aria, A. I., et al. (2016) Time evolution of the wettability of supported graphene under ambient air exposure. The Journal of Physical Chemistry C, 120(4), pp. 2215−2224.doi.org/10.1021/acs.jpcc.5b10492.
- Amadei, C. A., et al. (2014) Time dependent wettability of graphite upon ambient exposure: the role of water adsorption. The Journal of Chemical Physics, 141, p. 084709.doi.org/10.1063/1.4893711.
- Banhart, F., et al. (2011) Structural defects in graphene. ACS Nano, 5(1), pp. 26−41.doi.org/10.1021/nn102598m.
- Ma, J., et al. (2011) Adsorption and diffusion of water on graphene from first principles. PHYSICAL REVIEW B: Covering Condensed Matter and Materials Physics, 84(3), p. 033402.doi.org/10.1103/PhysRevB.84.033402.
- Werder, T., et al. (2003) On the water−carbon interaction for use in molecular dynamics simulations of graphite and carbon nanotubes. The Journal of Physical Chemistry B, 107(6), pp. 1345−1352.doi.org/10.1021/jp0268112.
- Song, Y., et al. (2021) Graphene transfer: paving the road for applications of chemical vapor deposition graphene. Small, 17(48), p. 2007600.doi.org/10.1002/smll.202007600.
- Chen, Y., et al. (2015) Growing uniform graphene disks and films on molten glass for heating devices and cell culture. Advanced Materials, 27(47), pp. 7839−7846.doi.org/10.1002/adma.201504229.
- Liu, X., et al. (2007) Influence of substratum surface chemistry/energy and topography on the human fetal osteoblastic cell line hFOB 1.19: Phenotypic and genotypic responses observed in vitro. Biomaterials, 28(31), pp. 4535−4550.doi.org/10.1016/j.biomaterials.2007.06.016.
- Lim, J. Y., et al. (2011) Optimizing the osteogenic potential of adult stem cells for skeletal regeneration. Journal of Orthopaedic Research, 29(11), pp. 1627−1633.doi.org/10.1002/jor.21441.
- Lim, J. Y., et al. (2008) Surface energy effects on osteoblast spatial growth and mineralization. Biomaterials, 29(12), pp. 1776−1784.doi.org/10.1016/j.biomaterials.2007.12.026.
- Kato, R., et al. (2020) High-precision thickness control of ice layer on CVD grown bilayer graphene for cryo-TEM. Carbon, 160, pp. 107−112.doi.org/10.1016/j.carbon.2020.01.010.
- Li, Z., et al. (2016) Water protects graphitic surface from airborne hydrocarbon contamination. ACS Nano, 10(1), pp. 349−359.doi.org/10.1021/acsnano.5b04843.
- Shi, Z., et al. (2008) Crystal structure of Escherichia coli PNPase: Central channel residues are involved in processive RNA degradation. RNA, 14(11), pp. 2361−2371.doi.org/10.1261/rna.1244308.